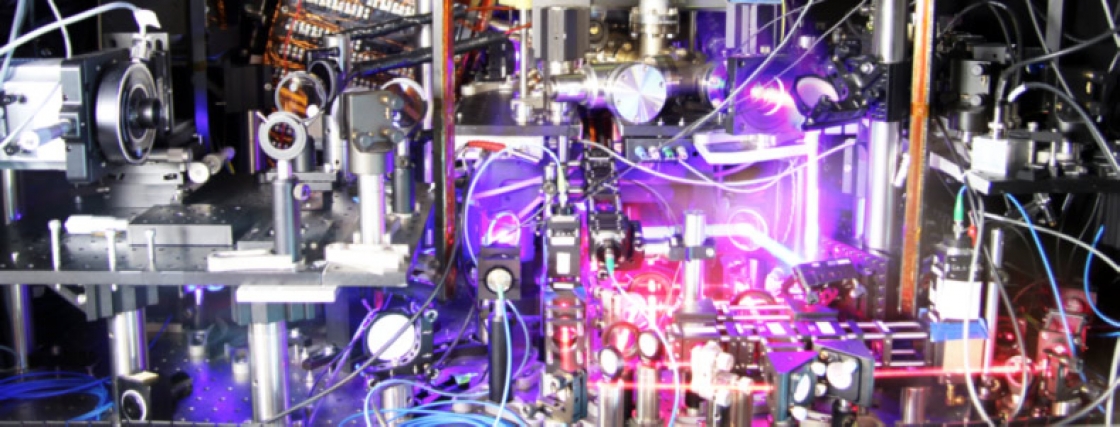
Strontium apparatus.
Our group explores many facets of ultracold strontium (Sr), emphasizing precision measurement and quantum state engineering and manipulation of atomic states. The group has achieved exquisite technical control via precision stabilization of lasers and the realization of ultracold atoms in optical lattices. Early on, we focused on precision measurements of Sr electronic transitions, which occur at optical frequencies, to explore the possibility of developing an optical atomic clock. This intense research effort has resulted in the success of demonstrating the Sr optical clock as the most stable and one of the most accurate clocks in the world. More recently, this research has found unique perspectives for the understanding of many-body quantum systems and their dynamics, as well as the exploration of quantum information science based on alkaline earth atoms. The preparation of novel quantum matter will allow us to continue to push the frontier for measurement science. Many-body quantum systems will advance the fundamental limit for precision while providing new opportunities for us to test basic physical principles. On the other hand, development of these advanced measurement capabilities will permit a full characterization of microscopic interactions and macroscopic system properties, leading to systematic understandings of new quantum materials.
Optical Atomic Clock
Our group has worked over a decade on the design, implementation, and enhancement of the world’s most precise and accurate optical atomic clock based on neutral atoms. The Sr-lattice clock is also the most stable optical atomic clock in the world. Its precision timekeeping mechanism is based on a narrow electronic transition in Sr atoms trapped inside an optical lattice, which is designed to separate the atomic internal and external degrees of freedom during clock measurement.
The clock’s accuracy is a measure of how well it keeps time against external perturbations and varying experimental parameters. Its precision reflects how close repeated time measurements are to one another. Related to the precision is the clock’s stability which is a measure of how long it has to operate to achieve its optimal accuracy. The Sr-lattice clock will neither gain nor lose a second in more than 200 million years of operation. It is approximately three times more accurate than the nation’s primary time and frequency standard, the NIST-F1 cesium (Cs) fountain atomic clock. Two independent Sr clocks are now running in our group, each achieving a record-level stability of a few parts in 1016 over a 1 s measurement time. The Sr-lattice clock’s optical transition has a much higher frequency than the microwave clock transition in Cs.
Our group has explored both the fermionic (87Sr) and bosonic (88Sr) isotopes for optical clocks. For 88Sr, we proposed a new clock scheme employing two lasers (and three atomic levels) to create a unique energy level transition that otherwise would not occur in nature. The new transition was very narrow and stable, an ideal combination for an atomic clock. For 87Sr, systematic characterizations of the nuclear spin (9/2) states and the related Stark and Zeeman effects have been carefully performed. This research enabled removing a major systematic uncertainty for the determination of the clock transition frequency. Interestingly, the combination of the metastable electronic states for the clock transition and the nuclear spins allows an efficient mechanism for quantum information processing. Also, the SU symmetry emerges in the nuclear spin degrees of freedom since both the clock states (1S0) and triplet (3P0) have electronic angular momentum J=0. All nuclear spin states are thus degenerate due to the lack of hyperfine structure. The presence of SU(N) symmetry is equivalent to the requirement that scattering between two atoms is independent of the nuclear spins. We thus have an opportunity to investigate, probe, and characterize SU(N) orbital quantum magnetism in an ultracold gas of Sr atoms using a state-of-the-art optical lattice clock.
As part of ongoing efforts to enhance the performance of the Sr-lattice clock, our group discovered in 2008–2009 that colliding fermions were causing frequency shifts in the optical clock. Until this unexpected discovery, most researchers assumed that there would be no systematic effects caused by collisions if fermionic Sr atoms were cooled to sufficiently low temperatures. This assumption was grounded in the laws of quantum mechanics, which mandate that identical fermions cannot get close enough to one another to actually collide. However, the exquisite precision attained by the optical lattice clock soon discovered that while these collisional effects were indeed small, they were not negligible for building the best clocks.
After a few years of intense work on this subject, the mystery of the colliding fermions has been unraveled. We have by now uncovered a unifying picture that can account for all of the observed frequency shifts in optical lattice clocks under different configurations. Not only did this study lead to an unprecedented characterization of collision frequency shift at an uncertainty below 1 x 10-18, it also helped launch an exciting new research direction, in collaboration with the Ana Maria Rey theory group. Strong interactions in a quantum system can give rise to novel dynamics conditioned upon the number of particles and the spatial dimension they are confined within. With the exquisite measurement precision, our optical lattice clock is being used to probe a strongly interacting quantum many-body system at the few-particle and many-particle limits, with dynamics that are beyond the mean-field description. The advance of the lattice clock performance and the study of many-body quantum physics are now intimately connected in our laboratory.
To help reach this exciting regime, we have developed the world’s most stable laser that can maintain optical coherence over 10 s. This laser has permitted us to approach (within a factor of 2) the fundamental quantum projection noise limit for the clock operation with a thousand atoms. The resulting stability of the Sr-lattice clock is currently at 2 x 10-16 at 1 s. This improved stability has greatly facilitated our efforts to characterize the overall uncertainty of the Sr-lattice clock.
Quantum Information Science & Many-Body Physics
Our group has been collaborating with groups of Ana Maria Rey (JILA), Misha Lukin (Harvard), Andrew Daley (Pittsburg) and Peter Zoller (Innsbruck) to explore the use of precision and control we have achieved for an optical lattice clock on quantum information science and quantum many-body physics. These collaborations have already led to a comprehensive theoretical framework for an optical-lattice quantum computer based on alkaline earth metals such as Sr. We have extensively investigated the interplay of the nuclear spin with two long-lived electronic states (or clock states) of Sr. As opposed to alkali atoms, alkaline earth atoms have electronic and nuclear states that can be independently manipulated since they are not coupled. An understanding of this interplay opens the door to quantum information storage and processing. Thus far the "information-processing" collaboration has proposed solutions for the key problems of storing, addressing, and transporting qubits (information encoded on the nuclear spins of Sr atoms). It has also put forward the advantages of a computer design that would use (1) local processors (i.e., quantum registers) for maintaining coherence and (2) qubit-state-controlled quantum gates for managing communications between qubits.
These studies have also led to the prospective for research on spin-orbital physics and quantum magnetism using alkaline earth atoms embedded in optical lattices. Other interesting areas in quantum many-body physics include the study of SU(N) physics and a many-body spin system with strongly interacting, open, and driven dynamics. Strongly interacting quantum many-body systems are prevalent in science. They can be intractably complex, are technologically important, and provide new opportunities for pushing forward the frontier of scientific knowledge. On the other hand, optical atomic clocks offer a pristine environment already engineered for measurements at the highest levels of precision, and where resolution of quantum fluctuations has been achieved. We can thus chart a new course for the study of complex quantum systems by uniting these two scientific realms. Decoupled from the external atomic motion, the internal spin degrees of freedom of many atoms, i.e., their clock states typically used for timekeeping, develop correlated quantum dynamics when all other fluctuations of technical origin are suppressed. An optical clock can thus shed light on quantum magnetism in an open quantum system. Research along this line represents both the development of a new set of tools and a new perspective for exploring the intricacies of complex quantum systems.
Connection to Ultracold Molecules
Our work on Sr lattice clocks also connects to the field of ultracold molecules. Using precisely tuned laser light, we have been able to use optical Feshbach resonances to induce a gas of ultracold 88Sr atoms to thermalize.
Controlling atomic interaction strength by magnetic Feshbach resonances has led to explosive progress in studies of few- and many-body quantum systems. The optical analog, where a scattering resonance arises from photoassociation via an excited molecular state, can enable control with high resolution. Narrow intercombination lines in alkaline earth atoms offer reduced incoherent scattering rates. The OFR effect is important for alkaline earth atoms due to their lack of magnetic structure in the ground state.
Photoassociation is a good way to create a large number of ultracold Sr2 molecules that are all in the same quantum mechanical state. To make the molecules, we confine ultracold (2µK) 88Sr atoms in a one-dimensional “magic” wavelength optical lattice of 914 nm. Trapped 88Sr atoms are then induced to form excited Sr2 molecules with a weak red laser at 689 nm. The narrow transition (1S0→3P1) stimulated by this laser makes it possible to detect many excited molecular states with high resolution. It also favors the decay of the excited molecules into tightly bound and stable ground-state molecules. The ground-state Sr2 molecules can then be used for precision measurements to help us understand whether particular fundamental constants of nature (such as the proton/electron mass ratio mp+/me-) have time dependence.
Ultrastable Lasers
A central feature of our group’s optical atomic clock is the world’s most stable and “quiet” clock lasers ever built. Since 2004, this laser has evolved to become so stable that it can now detect and measure quantum fluctuations in the optical atomic clock and the group’s new quantum simulator. It is a stunning achievement capping eight years of precision laser development in the Ye labs.
Two of the most stable optical cavities demonstrated so far are (1) a 40 cm cavity in our group (at 698 nm) that uses a spacer made of ultralow expansion glass and mirror substrates made from fused-silica glass - The long cavity spacer helps reduce the fundamental thermal noise; (2) an optical cavity at 1.5 um made of a single crystal of silicon, which resulted from a close collaboration between our group and researchers from Physikalisch-Technische Bundesanstalt (PTB) of Germany.
These cavities now support laser stability of 1 x 10-16 at 1 to 1000 s and both demonstrate linewidth on the order of 30 mHz. This new generation of super-stable lasers has allowed us to demonstrate quantum projection noise limited optical lattice clocks. We are currently working with scientists from Vienna to use crystalline-based optical coatings to further suppress the cavity thermal noise.
Other related efforts in pushing the frontier of ultrastable lasers include the exploration of collective effects in Sr atoms such that the intrinsic atomic coherence is used to generate and support highly coherent radiation.
Pulse-timing Synchronization and Distribution
The Ye lab participates in the optical clock comparisons by transmitting their clock signals back and forth across a 3.5-km fiber-optic link between JILA and NIST. The group began investigating the remote transfer of ultrastable frequency signals nearly a decade ago in anticipation that rapid development of optical atomic clocks might render GPS-based signal transfer inadequate. The new signal transfer system is already realizing its promise. At NIST, an optical frequency comb serves as an intermediate clock gear to facilitate comparison of the 87Sr lattice clock with other optical atomic clocks at NIST. This arrangement precisely and accurately transfers clock signals, thanks to recent work by the our group on coherent optical phase transfer over fiber networks. The group relied on a method for detecting and removing phase noise in a fiber link — a necessity since the local fiber-optic link is buried under Boulder city streets, where it is subject to traffic noise and temperature variations. To solve these problems, we heterodyne a signal that has made a round trip through a double-pass actuator and the fiber link against a reference. They then feed information about fiber-link noise contained in the heterodyne beat back into the actuator, which cancels the noise in the clock signal. Over time, the researchers have improved the stability of signal transmission over the fiber link by a factor of a hundred, ensuring that time can now travel even more accurately than the new 87Sr lattice clock can measure it.